CRISPR-Cas: from mechanism to applications
Issue: Future Tech
09 August 2016 article
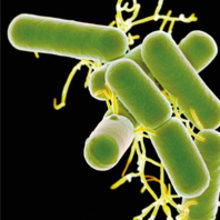
All organisms are exposed to parasites, so they evolved a range of different immune mechanisms to defend themselves. About 10 years ago, scientists discovered that bacteria and archaea, generally regarded as primitive organisms, have a sophisticated adaptive immune system, known as CRISPR-Cas (Clustered Regularly Interspaced Short Palindromic Repeats). The insights gained about this system led to applications in industry to protect bacterial species against their viral parasites. In addition, CRISPR-Cas has been turned into a versatile genome editing method that has the potential to treat human genetic diseases.
What is adaptive immunity?
There are many ways to categorise immune systems. One common distinction is whether immune systems are pre-programmed (innate) or re-programmable (adaptive). The best known example of adaptive immunity is the antibody response in vertebrates. Unlike innate immunity, adaptive immunity is acquired during the lifetime of the host and always results in a response that is highly specific towards the parasite that the host interacts with. For a long time it was presumed that adaptive immunity was restricted to higher organisms. Adaptive immunity in prokaryotes has been one of the most bewildering discoveries in microbiology.
The architecture of a CRISPR-Cas adaptive immune system
CRISPR-Cas systems consist of two key components. The first is the so-called CRISPR locus, which is located on the bacterial (or archaeal) genome. CRISPR loci function as a genetic memory of previous infections. They are composed of repeating sequences that are typically around 30 nucleotides in length. These repeating sequences (repeats) are separated by unique sequences of similar length. It is these unique sequences that matter most. These are the memory sequences, derived from parasite genomes, and they are referred to as ‘spacers’. Spacers in the CRISPR locus confer immunity against any parasites that carry a matching sequence. The sequence information stored in the CRISPR locus is used by the second key element of CRISPR-Cas systems, the Cas proteins. Cas (for CRISPR-associated) proteins are often encoded next to a CRISPR locus.
The three stages of CRISPR-Cas
How do CRISPR-Cas systems work? In a nutshell, CRISPR-Cas systems capture pieces of DNA from a parasite (for example, a virus), integrate this into the CRISPR locus, and use this information to destroy the parasite when it infects again. This process is generally described as having three (more or less) independent stages. The first is the ‘Adaptation’ stage, which occurs immediately upon infection with a novel parasite. During ‘Adaptation’, dedicated Cas proteins capture a piece of parasite DNA and integrate this into the CRISPR locus, where it forms a novel spacer. Next, the ‘Expression’ stage takes place, during which the entire CRISPR locus is copied into an RNA molecule. This long RNA transcript is cleaved by dedicated ribonucleases to generate small crRNA molecules that contain only a single spacer sequence. A single crRNA forms a complex with one or multiple Cas proteins. This complex is one of the key functional modules of the CRISPR-Cas immune system, and functions analogous to an antibody in parasite detection. In the event of re-infection with the same parasite, the ‘Interference’ stage is triggered. During this stage, Cas-crRNA complexes bind and cleave the genome of the parasite, which results in host immunity.
Two classes of CRISPR-Cas systems
CRISPR-Cas systems are highly diverse, and have been classified into two main classes, and several types and subtypes. One of the most obvious differences is that Class 1 systems encode many more Cas proteins compared with Class 2 systems. Unlike Class 1 systems, Class 2 Cas-crRNA complexes contain only a single Cas protein – the most famous example being Cas9 – and because of their relatively low complexity, Class 2 systems are uniquely suited for genome engineering applications.
Genome engineering
Introducing specific mutations into a genome has long been extremely challenging. Our detailed understanding of CRISPR-Cas systems has facilitated the development of a CRISPR-Cas9-based genome editing technology that makes it much more straightforward to introduce any mutation of interest into the genome of our favourite study organism. Some first studies indicate that this technology can be used to cure human diseases that are caused by specific genetic mutations. What makes CRISPR-Cas9 systems so suitable for genome editing? Firstly, a key feature of crRNA-Cas9 complexes is that they will specifically interact with DNA sequences that are complementary to the crRNA. Secondly, this specificity can be readily adjusted by changing the crRNA sequence, which provides extreme control over the crRNA-Cas9/DNA interaction. Thirdly, Cas9 will cleave the corresponding sequence, resulting in a DNA break, which is an essential step in the genome editing process. The double-stranded break will be repaired by a DNA repair pathway. Depending on the method that is used, DNA will be repaired through the non-homologous end joining (NHEJ) pathway, or the homology-directed repair (HDR) pathway. NHEJ will result in higher efficiency of mutation incorporation, but with little control over the mutations that are introduced, while HDR provides full control over the mutations but with lower efficiency.
THIS PROTEIN (PURPLE) IS USED IN GENOME ENGINEERING TO CUT DNA. IT USES A GUIDE RNA SEQUENCE (GREEN) TO CUT DNA (BLUE) AT A COMPLEMENTARY SITE. THE CUT OF DNA IS SHOWN IN YELLOW. THE PROCESS OF INSERTING A FRAGMENT OF DONOR DNA (RED) IS SHOWN AT LOWER CENTRE, WITH A GENETICALLY MODIFIED LABORATORY MOUSE.
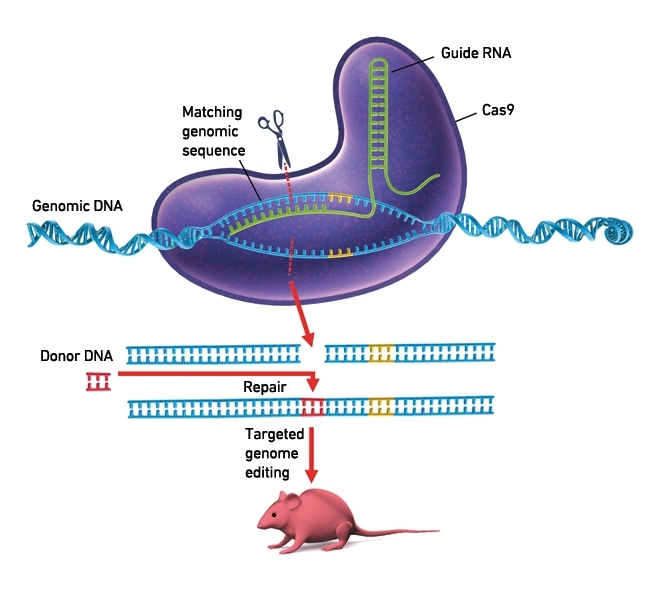
CRISPR-Cas to protect bacterial species in industry
Much of the initial interest in CRISPR-Cas came from the industry working with lactic acid bacteria. For example, yoghurt production can fail due to virus infections during the fermentation, which leads to product downgrading or loss. In the food industry, there is great interest in bacterial strains with naturally evolved resistance, and CRISPR provides an ideal system to generate strains that are more robust. However, these applied aspects of bacterial culture protection naturally lead to questions concerning the evolution and ecology of CRISPR-Cas systems. It was recently demonstrated that mounting a CRISPR-Cas immune response is associated with a fitness cost for the host. This may help to explain why only half of all bacteria have these sophisticated adaptive immune systems. Understanding the costs and benefits of CRISPR-Cas across different environments will help to design optimal defence strategies for bacterial species.
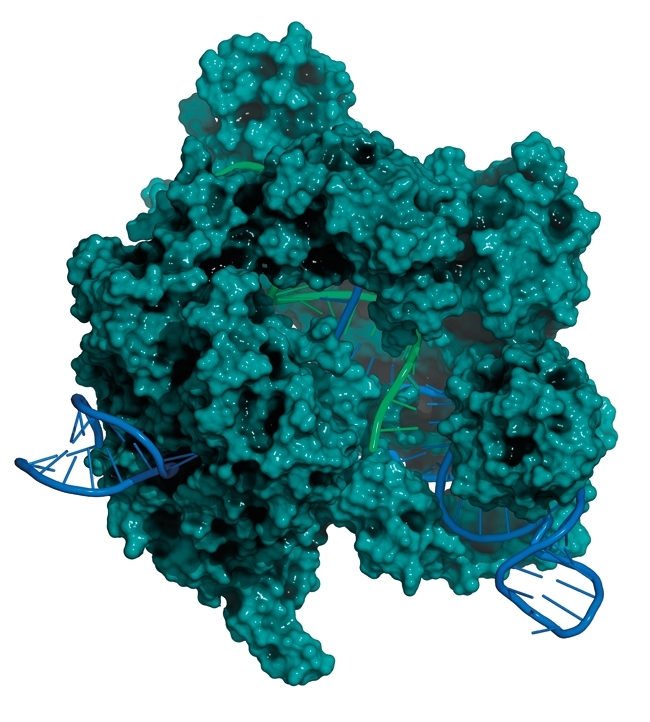
CRISPR-Cas and antibiotic resistance
Antibiotic resistance genes often move horizontally between bacterial species, for example through plasmid conjugation. Bacterial immune systems may limit such spread, since they can form a barrier for incoming DNA. The interaction between CRISPR-Cas and antibiotic resistance is unclear; some studies support the hypothesis that CRISPR-Cas blocks the spread of resistance genes, whereas other studies found no evidence for this. Understanding the short- and long-term implications of CRISPR-Cas is key if we are to predict and manipulate the spread of antibiotic resistance.
The discovery of the CRISPR-Cas system led to a sea of change in the microbiological sciences. Adjusting to the concept that ‘primitive organisms’, such as bacteria and archaea, had sophisticated adaptive immune systems was just the start. Since then, scientific endeavour has seen the number of potential uses for this primitive immune system, including genetic engineering with higher organisms, increase rapidly. In turn, the potential applications for this primitive microbial system are providing ethical questions that need to be addressed by society as the implications for the CRISPR-Cas system continue to emerge, especially within clinical settings.
EDZE WESTRA
University of Exeter, Penryn Campus, Penryn, Cornwall TR10 9EZ, UK
[email protected]
Image: Coloured scanning electron micrograph of Lactobacillus bacteria. Science Photo Library. Diagram of the process that occurs within the CRISPR-Cas9 gene editing complex. Gunilla Elam/Science Photo Library. CRISPR-Cas9 gene editing complex from Streptomyces pyogenes. Molekuul/Science Photo Library..