Breaking bottles: microbial degradation and remediation of PET plastic
Posted on November 24, 2020 by Jack Henderson
Microplastic pollution is currently at the forefront of public interest in environmental science, with widespread effort to curb plastic usage in order to mitigate environmental catastrophe. In this blog, Jack Henderson discusses the role microbes have to play in solving the plastic pollution problem.
Polyethylene terephthalate (PET) is the world’s most abundant synthetic polyester and one of the most popular plastic types. Widely used in everyday products from plastic bottles to clothes and textiles, PET holds tremendous value in contemporary society through affordable clothing and lightweight packaging. However, accumulation of PET wastes is a serious environmental hazard, threatening aquatic ecosystems and global food chains. Unfortunately, the same properties which makes PET a valuable asset – durability, pliability, hydrophobicity and resistance to degradation – lends to its role as a persistent pollutant which chokes our aquatic ecosystems.
Microplastics – defined as particles of plastic less than 5 mm in diameter – have brought plastic pollution back into the forefront of public conversation. Microplastics have become ubiquitous in the environment. Found everywhere from the Arctic to the Mediterranean, plastics have a profound effect on marine wildlife. Microplastic rain has also been observed, further polluting our terrestrial ecosystems.
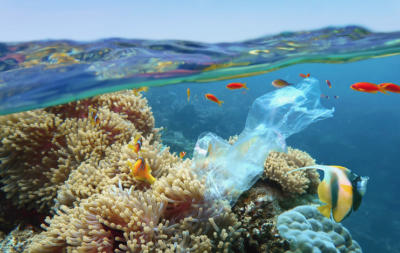
Accumulation of microplastics in the gastrointestinal tract of marine animals may cause blockage and/or inflammation, resulting in malnutrition and death. Microplastics may also accumulate and obstruct the gills of fish. The hydrophobic nature of plastic facilitates the transport of toxins, such as persistent organic pollutants, at a much higher concentration than the surrounding water. Additive chemicals such as dyes and flame retardants may also be toxic. Microplastics typically interact with species at the base of the food chain, threatening the stability of aquatic ecosystems. Accumulation of microplastics and their associated toxins may then impact human food sources with the full effect on human consumption currently unknown.
Microplastic pollutants are categorised as primary or secondary. Secondary microplastics are produced via fragmentation of macroplastics, such as a plastic bottle. Primary microplastics, on the other hand, are microplastics upon initial release. A major and emerging source of primary microplastics is the tiny plastic fibres which break off from synthetic clothing during washing and wearing. These microfibres – plastic fibres less than 5mm in length – wash directly into aquatic ecosystems after passing through the wastewater treatment system, which is currently unable to fully eliminate them. Studies have shown that 1 kg of synthetic clothing produces up to 1.5 million fibres per wash. It is estimated that microfibres account for up to 35% of primary microplastics that enter the ocean. PET is a key component in polyester fabrics, which is the most widely used synthetic fabric in the world.
Although PET is damaging to the environment, it is unreasonable and impractical to totally halt its use: PET is a valuable asset to many, especially those in low-income areas, providing affordable clothing and a means of transporting food and water. In order to solve global challenges, the solutions must be accessible to all. Therefore, although stewardship is important, development of smart recycling and remediation strategies is crucial in guiding sustainable use of PET.
Microbial degradation of PET utilises various hydrolases, collectively referred to PETases, to break the ester bonds of PET, resulting in the production of mono(2-hydroxyethyl) terephthalate (MHET) as a primary degradation product. Currently, PET degradation has been limited to a few species of mainly thermophilic bacteria. In 2016, a novel PET degrading bacterium, Ideonella sakaiensis was isolated from a PET recycling plant in Japan [5]. Unlike previously characterised PET degraders, I. sakaiensis was discovered to metabolise PET in moderate temperatures. Additionally, I. sakaiensis utilises a unique enzyme (MHETase) to uptake and metabolise MHET, which is a major rate-limiting factor in PET degradation. In addition to several bacterial PET degraders, strains of the fungal species Fusarium have also been shown to degrade PET.
Although several PET degrading microbes have been discovered, the rate of degradation is slow: I. sakaiensis takes 6 weeks to breakdown a thin film of PET. Highly crystalline PET, used in products such as water bottles, is particularly resistant to degradation. The slow rate of PET degradation is a major roadblock in the development of effective and affordable recycling solutions.
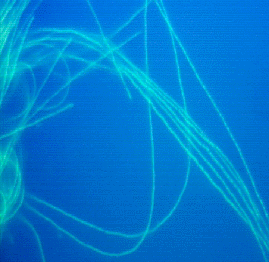
Protein engineering may hold the key for increasing the rate of PETases. This approach has succeeded in increasing the I. sakaiensis PETase activity by 530% at 30°C. However, the rate of degradation increases 14-fold when incubated at 40°C. This leads on to the next approach: increasing the temperature. Increasing temperature breaks the hydrogen bonds between polymer chains, allowing increased access to the ester linkages and thus increasing the rate of degradation while also facilitating the degradation of high crystallinity PET. Thermophilic PET degraders such as Thermobifida fusca demonstrate far greater PET degradation ability when compared to I. sakaiensis when incubated at temperatures over 50°C. An engineered mutant of the T. fusca PETase was able to degrade 90% of bottle grade PET in just 10 hours at 72°C. This research is promising for the future of PET recycling: increasing the temperature allows higher crystallinity PET to be degraded faster. Although more efficient, temperature increases have an impact on the economic viability of recycling, as well as having possible sustainability impacts from a climate change perspective. The efficiency of PETase can also be increased by simultaneous degradation of MHET: a recent study demonstrated that physically linking PETase and MHETase produced a tandem which was 6 times more efficient than PETase alone. This could facilitate more economic recycling of PET.
Currently characterised PET degraders represent an extremely limited group of organisms, however, there is good evidence that there may be many more species which are able to degrade PET! Using a machine learning model, Danso and colleagues screened genome and protein sequence databases for potential PETases. This study revealed that PETases may be more abundant than previously thought, with over 500 potential PETases previously undiscovered. The discovery of novel PETases with increased rate and efficacy against high crystallinity PET is imperative, as it allows protein engineering to advance as well as allowing possible augmentation of highly polluted areas, such as the Great Pacific Garbage Patch, for bioremediation of PET wastes. Their research found the highest abundance of possible PETases in a metagenome of crude oil-contaminated sediment, inferring a possible link between hydrocarbonoclastic (hydrocarbon degrading) organisms and PET degradation. This potential link was further compounded when it was demonstrated that Pseudomonas aestusnigri, a well-characterised hydrocarbonoclastic bacterium, degraded PET [10]. This link should be explored in more depth as it may provide a large, untapped pool of potential PETases.
Discovery of novel PET degrading organisms with enhanced PET degradation rate could be used to augment microbial communities in the wastewater treatment process, facilitating complete degradation of PET fibres before they are washed into the ocean, or applied to land. Alternatively, bioreactors could be integrated into the wastewater system to allow degradation of PET fibres. This may also allow the production of biogas from plastic pollution.
Continuing to innovate PET remediation and recycling solutions is in the best interest of the global ecosystem and global health as a whole. Microbial degradation of PET provides an opportunity to reduce the cost of recycling and thus facilitate the interception of polyethylene plastics before they enter the environment.
References:
Plastic rain is the new acid rain
Lu Y, Zhang Y, Deng Y, Jiang W, Zhao Y et al. Uptake and Accumulation of Polystyrene Microplastics in Zebrafish (Danio rerio) and Toxic Effects in Liver. Environ. Sci. Technol. 2016;50: 4054-4060.
Ma P, Wang MW, Liu H, Chen YF, Xia J. Research on ecotoxicology of microplastics on freshwater aquatic organisms. Environmental Pollutants And Bioavailability. 2019;31:131-137.
De Falco F, Di Pace E, Cocca M, Avella M. The contribution of washing processes of synthetic clothes to microplastic pollution. Sci Rep. 2019 DOI: 10.1038/s41598-019-43023-x.
Yoshida S, Hiraga K, Takehana T, Taniguchi I, Yamaji H et al. A bacterium that degrades and assimilates poly(ethylene terephthalate). Science. 2016;351:1196-1199.
Son HF, Cho IJ, Joo S, Seo H, Sagong HY et al. Rational Protein Engineering of Thermo-Stable PETase from Ideonella sakaiensis for Highly Efficient PET Degradation. ACS Catal. 2019;9:2519-3526.
Tournier V, Topham CM, Gilles A, David B, Folgoas C et al. An engineered PET depolymerase to break down and recycle plastic bottles. Nature. 2020;580:216-219.
Knott BC, Erickson E, Allen MD, Gadom JE, Graham R et al. Characterization and engineering of a two-enzyme system for plastics depolymerization. PNAS. 2020;117:25476 – 25485.
Danso D, Schmeisser C, Chow J, Zimmermann W, Wei R et al. New Insights into the Function and Global Distribution of Polyethylene Terephthalate (PET)-Degrading Bacteria and Enzymes in Marine and Terrestrial Metagenomics. Appl. Environ. Microbiol. 2018;84:,1-13.
Bollinger A, Thies S, Knieps-Grünhagen E, Gertzen C, Kobus S et al. A Novel Polyester Hydrolase From the Marine Bacterium Pseudomonas aestusnigri – Structural and Functional Insights. Front. Microbiol. 2020;11:114 .